Evolution
Intelligent Design
Life Sciences
Hailed as the “Next Darwin,” MIT’s Jeremy England Sheds Little Light on Life’s Origins
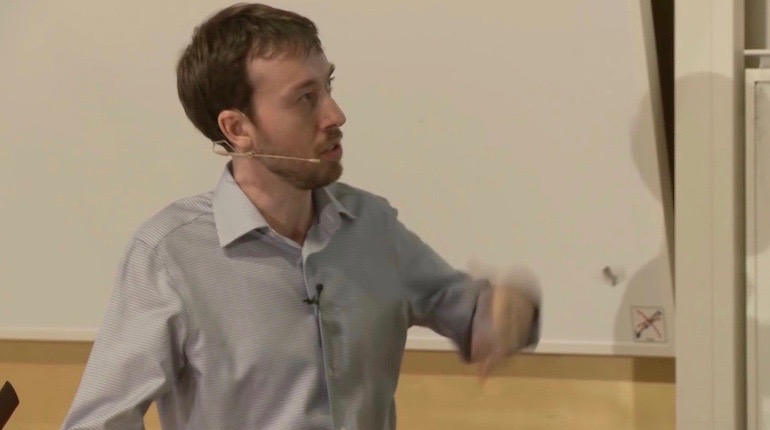
A rising star at the intersection of physics and biology is MIT professor Jeremy England. He has been described as the “next Charles Darwin,” and he is credited with a new theory of life based on physics. England’s proposals draw from theories of non-equilibrium thermodynamics related to systems driven far from equilibrium. The theoretical framework originated with the pioneering research of Ilya Prigogine on self-organization, and it was further developed by such evolutionary theorists as Daniel Brooks and E. O. Wiley. England is a top-level scientist, and his research will undoubtedly yield valuable insights into non-equilibrium systems. However, in applying his findings to life, he will face the same barriers as his predecessors:
- Fundamental differences exist between self-organizing systems and biological structures and processes.
- The origin and development of life required preexisting machinery to process energy and external sources of information to drive required chemical reactions and to manufacture biological structures.
England’s approach is to relate the tendency for any driven system to transition from one class of states (e.g., chemicals on the early earth) into another (e.g., life) to the amount of total entropy that is produced in such conversions. Specifically, the irreversibility of the transition between classes grows with the sum of the internal entropy increase and the entropy (heat) released into the environment. He also demonstrates that transformations between states are most likely where energy is most reliably dissipated — energy output is high and fluctuations are low.
Building on these insights, England’s lab simulated a network of interconnected chemical reactions with added external forcing. The thermodynamic forcing altered the dynamics of specific reactions. A real-world example of such forcing would be constantly adding a chemical that could accelerate or slow a particular reaction, depending on whether it was a reactant or a product. Other examples would be supplying UV light or shock waves. England ran simulations where the effect of the forcing depended on the configuration of the matter (i.e., the nature of interactions and the relative abundance of molecules) and only a few configurations resulted in strong forcing effects. His experiments demonstrated that the system often moved into the states that maximized the forcing and thus the efficiency of dissipating energy. In other words, the matter at times self-organized (emerged) into configurations that were “fine-tuned” for extracting energy from the environment.
England and his colleagues have drawn parallels between these results and possible scenarios for the development of life. They propose that similar self-organizing processes could have driven life’s origin, such as producing self-replicating molecules. However, such claims face two major problems. First, the underlying equations imply that any class of states will tend toward an increase in the total entropy. However, the coalescing of simple chemicals into a cell would have resulted in a decrease in total entropy — the internal entropy would have decreased, and energy (e.g. heat) would have been extracted from the environment. Therefore, any realistic collection of molecules would have moved away from a life-permitting state.
The story is actually more complicated. The underlying equations used by England relate the entropy production of a system transforming from initial “macrostate” I to final state II, while undergoing some driving force, to the measure of irreversibility. This measure is defined as the probability of the forward transition divided by that of the reverse transition. However, the driving force, such as a piston compressing a cylinder, in the reverse transition is assumed to run backwards. In the case of the piston, the reverse direction would correspond to the piston decompressing or moving outwards. The challenge comes in envisioning on the early earth the meaning of external influences, such as sunlight, running backwards. However, such complications do not change the results. Ignoring all external forcing, any system would move in the opposite direction of forming the first cell. And, the added effect of considering external energy sources is simply to accelerate the breakdown of life’s precursors, such as RNA. To be more rigorous, any system driven from equilibrium by some external energy source will result in the “dissipation function” throughout the system to, on average, maintain a positive value. The value of the dissipation function closely relates to the rate of production of entropy, which means that the entropy will never dramatically decrease in a driven system as required for life’s origin.
Second, England’s model assumes that external driving forces directly affect the chemical reactions defining a system, and the matter in turn reconfigures to better resonate with the driving forces. However, in life, cellular structures drive and interconnect reactions that would not happen naturally. Most significantly, complex cycles process energy from chemicals in the environment or from sunlight and store it in high-energy molecules, such as ATP. Other cellular machinery then connects the breakdown of those molecules to specific cellular reactions, providing the needed energy and catalytic assistance. As a result, no direct physical connection exists between external driving forces and most cellular reactions, since the high-energy molecules act as intermediaries. Meanwhile, the driving and coupling of reactions takes place through enzymes binding to target molecules as a consequence of the former’s precise 3D shapes, and their shapes result from the information in their amino acid sequences. This information determines every cellular activity.
At a more fundamental level, England’s research is focused on the wrong question. Origin-of-life theories are not helped by identifying processes that efficiently dissipate energy. Instead, life requires machinery that can harness the energy from some source and then transform it into a form that is useful for cellular operations. As an analogy, an open camp fire releasing heat will not help transport cargo across the country. Instead, a steam engine is needed to access the energy from burning fuel and redirect it into mechanical energy to turn the train wheels. No self-organizing system will accomplish the equivalent goal in a cell. For, even the simplest cellular engines would require complex structures coupled to a highly-orchestrated network of chemical reactions.
The overarching challenge is that the number of possible configurations and interactions of molecules that could exist in even simple cells is astronomical. One recent study estimated the total for a single yeast cell to exceed 10 to the power of 10 billion. The number that would allow the simplest cell to function is undoubtedly quite large, but the ratio of viable options to all possible ones must be miniscule — a proverbial needle in a haystack the size of our galaxy. The first cell required the selection of the correct set of reactions with their corresponding enzymes, which amounts to at least tens of thousands of bits of information appearing at once. If the cell were missing even one essential molecule, it would quickly break apart and decompose back into simple chemicals. Therefore, the answer to life’s origins cannot come for any self-organizing process but, rather, from preexisting information.
Similar challenges exist in applying physics models to the evolution of life. England and his team have been very modest in their claims, describing their research as only having “possible analogies” to evolution. However, others are already jumping on the possibility of adding his concepts to the Extended Evolutionary Synthesis. Writing for Quanta Magazine, Natalie Wolchover comments:
Having an overarching principle of life and evolution would give researchers a broader perspective on the emergence of structure and function in living things, many of the researchers said. “Natural selection doesn’t explain certain characteristics,” said Ard Louis, a biophysicist at Oxford University, in an email. These characteristics include a heritable change to gene expression called methylation, increases in complexity in the absence of natural selection, and certain molecular changes Louis has recently studied.
If England’s approach stands up to more testing, it could further liberate biologists from seeking a Darwinian explanation for every adaptation and allow them to think more generally in terms of dissipation-driven organization. They might find, for example, that “the reason that an organism shows characteristic X rather than Y may not be because X is more fit than Y, but because physical constraints make it easier for X to evolve than for Y to evolve,” Louis said.
Unfortunately, dissipation-driven theories face the same limitations as other self-organization models. The development of complex adaptations requires the input of large amounts of information to specify new biological structures and their accompanying metabolic pathways. For instance, in desert environments, CAM photosynthesis is more efficient than normal photosynthesis. However, the transition requires multiple coordinated alterations. The timescales needed for random mutations to bring the CAM system sufficiently online to provide an advantage would be prohibitively long. And, nature could not anticipate the new design would better fine-tune the plant to processing sunlight in the new environment and then target the needed changes. Like all attempted additions to evolutionary theory, England’s insights could prove valuable in explaining minor alterations, but they will provide no help in explaining true innovations.