Evolution
Intelligent Design
Life Sciences
Researchers Highlight Logistics Nightmare Facing Chromosome Controls
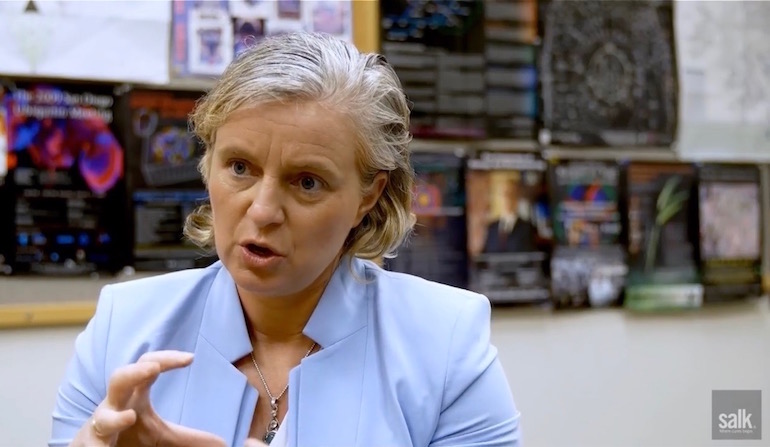
No human has ever faced a logistics problem this big. Consider that you have enough DNA in your body to reach Pluto, and it all has to be quickly readable and accessible from a volume one thousandth of a millimeter in diameter. The Library of Congress looks tame by comparison. Oh, and let us not forget — all that DNA has to be copied, separated, and repackaged quickly every time a cell divides. This all has to work in an organism able to pole vault, high dive, or fly in a wingsuit.
One sure marker of intelligent design is that the closer you look at a designed object, the more complex it becomes. That is certainly true of chromosomal packaging. Since the discovery of DNA, scientists have been able to peer closer and closer over decades. Each time they do, they find more complications to the logistics problems a cell faces. Yet DNA operations work remarkably well. If they didn’t, we wouldn’t be around to know otherwise.
Un-Simplifying Chromatin
Molecular biologists figured out in vitro (outside of living cells) that DNA wraps around balls of protein called chromatin, forming nucleosomes. They envisioned nucleosomes coiling and supercoiling into higher-order structures of increasing diameter, till the familiar chromosomes took shape. The chromatin balls were thought to be the same size (about 11 nm). A new finding announced by the Salk Institute now shows that they vary considerably in diameter, providing another level of flexibility for packing — and consequently a new headache for logistics.
While solving a “longstanding biological mystery of DNA organization,” the researchers illuminated a higher-order structure of DNA in the nucleus, with functional consequences. In a video clip (at the top of this post), Clodagh O’Shea, clearly excited by the findings, explains that each cell, while having the same copy of the genome, reads and interprets it for its role in the body. The organizational information must be inherited at mitosis as well as the sequence information.
Horng Ou then explains how the Salk team was able to image chromatin in the natural state within intact cells. “Life occurs in 3D, right,” O’Shea continues; “form gives way to function.” With the new imaging techniques, the team can begin to see DNA the way enzymes see it. Their results were published in Science:
What O’Shea’s team saw, in both resting and dividing cells, was chromatin whose “beads on a string” did not form any higher-order structure like the theorized 30 or 120 or 320 nanometers. Instead, it formed a semi-flexible chain, which they painstakingly measured as varying continuously along its length between just 5 and 24 nanometers, bending and flexing to achieve different levels of compaction. This suggests that it is chromatin’s packing density, and not some higher-order structure, that determines which areas of the genome are active and which are suppressed. [Emphasis added.]
So it is not some “cartoon drawing” type of organization, as has been taught in textbooks, but a complex space of voids and dense parts, hills and valleys that makes for a much more efficient packing density on the one hand, with tighter controls on access for enzymes and transcription factors. O’Shea sums it up:
“We show that chromatin does not need to form discrete higher-order structures to fit in the nucleus,” adds O’Shea. “It’s the packing density that could change and limit the accessibility of chromatin, providing a local and global structural basis through which different combinations of DNA sequences, nucleosome variations and modifications could be integrated in the nucleus to exquisitely fine-tune the functional activity and accessibility of our genomes.”
Controlling Gene Access
How does a cell decide which combination of genes is needed for its function in the body? An entry in the Oxford Science Blog asks, “How does a cell know which combination of the 20,000 genes it should activate to produce its specific toolkit?” Look in the formerly named junk DNA for clues:
The answer to this question may be found in the pieces of DNA that lie between our protein-producing genes. Although our cells contain a lot of DNA, only a small part of this is actually composed of genes. We don’t really understand the function of most of this other sequence, but we do know that some of it has a function in regulating the activity of genes. An important class of such regulatory DNA sequences are the enhancers, which act as switches that can turn genes on in the cells where they are required.
Switches are not junk. To show that they have important functional roles, the team looked at one enhancer called CTCF.
Researchers have identified key proteins that appear to define and help organise this domain structure. One such protein is called CTCF, which sticks to a specific sequence of DNA that is frequently found at the boundaries of these domains. To explore the function of these CTCF boundaries in more detail and to investigate what role they may play in connecting enhancers to the right genes, our team studied the domain that contains the α-globin genes, which produce the haemoglobin that our red blood cells use to circulate oxygen in our bodies.
Firstly, as expected from CTCF’s role in defining boundaries, we showed that CTCF boundaries help organise the α-globin genes into a specific domain structure within red blood cells. This allows the enhancers to physically interact with and switch on the α-globin genes in this specific cell type. We then used the gene editing technology of CRISPR/Cas9 to snip out the DNA sequences that normally bind CTCF, and found that the boundaries in these edited cells become blurred and the domain loses its specific shape. The α-globin enhancers now not only activate the α-globin genes, but cross the domain boundaries and switch on genes in the neighbouring domain.
Maintaining Chromosome Number
How do cells guarantee that 23 chromosomes will end up in each daughter cell? Researchers at Queen Mary University of London tackled that logistics question. They offer a factoid that indicates the precision of quality-control mechanisms: “Cells divide at least a billion times in the average person, usually without any problem.” Nevertheless, rare abnormalities do occur, as in the case with Down syndrome, when the wrong number of chromosomes (aneuploidy) results.
Using high resolution microscopes to video the inner workings of live human cells, Dr Draviam and her colleagues at the University of Cambridge (UK) and the European Molecular Biology Laboratory in Heidelberg (Germany), discovered that two proteins — Aurora-B kinase and BubR1-bound PP2A phosphatase — act in opposition to each other, adding or removing phosphate groups respectively, to correctly control the attachment of microtubules to the chromosomes.
Co-author Duccio Conti, who is Dr Draviam’s PhD student, said: “We found that a balance between Aurora-B kinase and BubR1-bound phosphatase is important to maintain correct chromosome numbers in human cells.”
Conclusions
At points in these articles, the authors hit pay dirt when they went looking for function. That’s what design science does: rather than being a science-stopper, as critics still sometimes say, it motivates scientists to find the reasons for things. Systems that solve major logistics problems, control access, and maintain structures speak of intelligent causes that have a purpose (a function) and organize parts to achieve that function. There’s hardly a better example in the world of that than what goes on in the nucleus of every cell.