Intelligent Design
Allostery: How Cells Do Remote Control
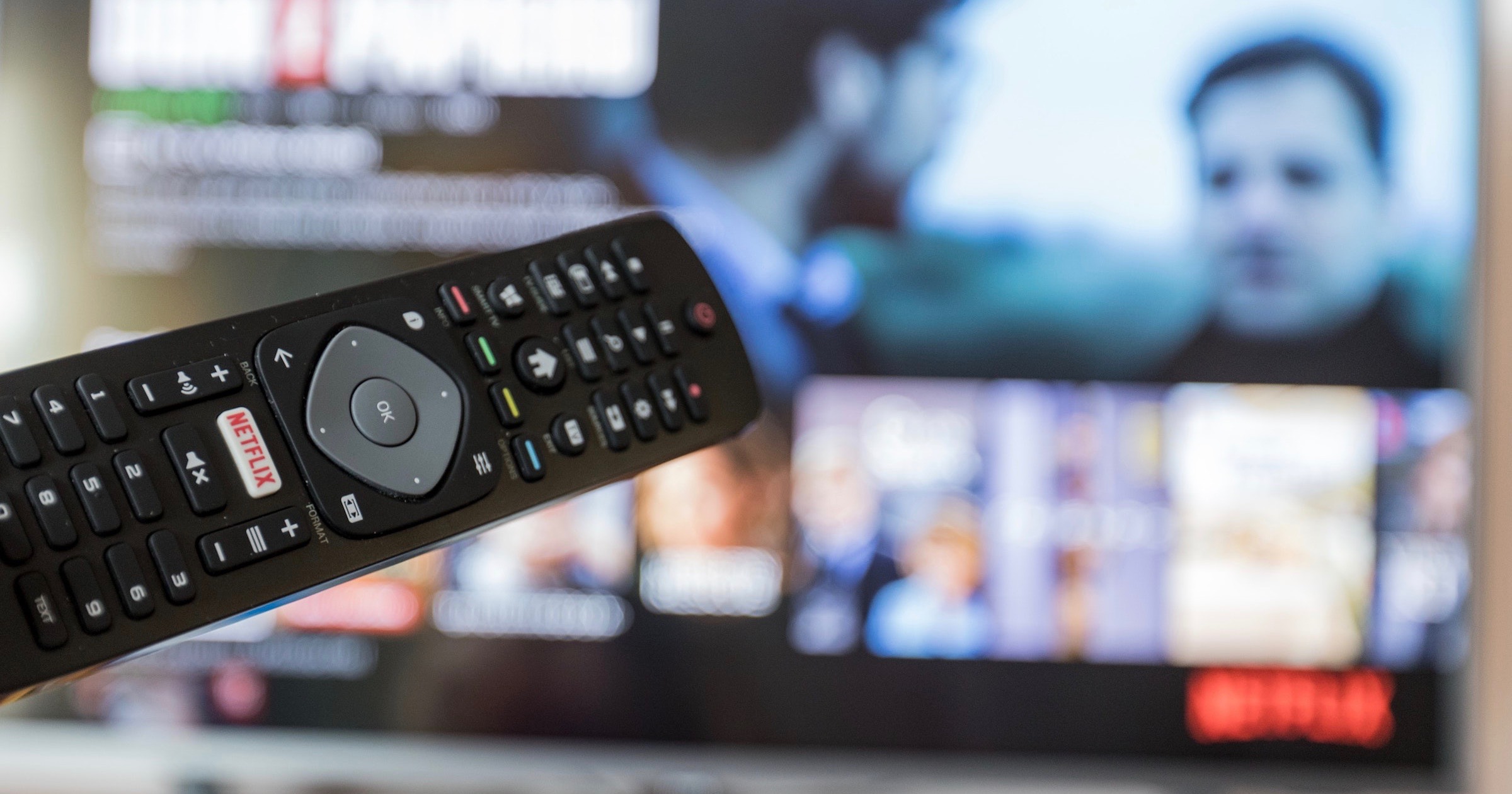
To a space alien visiting Earth, it might seem like magic: the human flips a switch here, and a light goes on over there! The more distant the light, the more magical it seems. Is this “spooky action at a distance” that physicists wonder about when discussing gravity and quantum mechanics? No; the alien quickly learns that there are physical connections between them that were designed for this ability: the switch was meant to turn on the light. Traditional lighting is wired physically, but smart lights can be controlled by wireless communications from a server (although they still need electrical wiring to the grid). The light and the switch did not “evolve” to connect; remote control shows that the signal and response required advance planning.
Human designers can get clever with remote control. At the 1933 Chicago World’s Fair, light from the star Arcturus, thought to be 40 light-years away at the time, was focused on photoelectric cells that triggered floodlights at the exposition grounds. Organizers boasted that light from the star had left in 1893 in order to launch the fair (Space.com). Today, it is routine for NASA engineers to send coded messages via radio waves to activate processes on spacecraft on Mars or even across the solar system. Now that’s foresight!
Cells perform remote control, too, using diverse methods. Since Evolution News addressed the subject of allostery four years ago, more interesting examples have been discovered. And like the 2016 example showed, remote control adds another layer of specificity to molecular machine design. “Allostery is a fundamental element of complex macromolecular function,” the authors of today’s first example explain, “representing the essential process by which events occurring at one site are transmitted to distal sites to regulate activity.” (Emphasis added.)
The Ribosome: Allostery in RNA, Too
Proteins were the first objects scrutinized for allostery. Allison Walker and three colleagues have now found that RNA can also do tricks with remote control. They say in PNAS1:
While multiple studies recognize that allostery contributes to complex function in RNAs such as riboswitches and the spliceosome, most studies of allostery focus on proteins. Thus, the capacity of RNA structures to support allosteric function remains underappreciated. Indeed, the fundamental discovery that small ribozymes populate unique and well-defined tertiary structures that support function and the more recent structure of the ribosome, revealing an active site composed entirely of RNA, reinforce the parity of protein and RNA structural and functional complexity.
They measured a “hierarchical organization” of parts inside the 23S RNA portion of the large ribosomal subunit “with near-independent groups of nucleotides forming physically contiguous networks within the three-dimensional structure.” They were able to demonstrate that two particular sectors of the RNA molecule “contribute to ribosome function” by showing that they “map to distinct ribosome activities” — performing remote control, in other words, just like allosteric proteins can. “RNA enzymes appear to share a common internal logic of interaction and assembly.” How’s that for ID thinking! Logical. Too bad they attribute the precision of function to sectors “co-evolving” within “functional constraints.” In other words, the sectors of the 23S RNA did not evolve, because changes would have broken its primary function: “the templated polymerization of α-amino acids.” Anyone know of templates that evolved by chance? They are speaking of how the ribosome matches amino acids attached to transfer RNAs to the codons on the messenger RNA template coming in for translation; watch the animation here at minute 1:30. Mutations are not likely to improve a process like that!
Bypassing the evolutionary language in this paper, what is most noteworthy is the extraordinary degree of allosteric cooperation in this RNA molecule. Remember that RNAs tend to be floppier than proteins. And yet the high-fidelity cooperative action between the RNA and protein parts in the ribosome require allostery over vast distances (on the cellular scale). This is astonishing. How can they believe this level of remote control just happened?
The size and complexity of its structure and the diversity of covalent and noncovalent interactions required to execute 10 to 20 translation cycles per second with high fidelity demand the coordinated interactions of bases that span more than 390,000 Å of molecular surface. But how does the ribosome actually work? How is molecular information transferred between functional centers? What are the allosteric pathways and conduits of communication, and how do specific residues in the structure contribute to this communication? In this work, we apply a global coevolution method (SCA) to show that, despite differences in fundamental chemistry, RNA and protein appear to use a common logic of interaction and assembly.
Assembly! It’s not just the interaction to consider, but the assembly, too. The ribosome parts had to be translated first, and then the building blocks, consisting of two very different molecules (RNA and protein), have to be assembled to work. And that work involves remote controls, where “molecular information” is “transferred between functional centers” by means of “allosteric pathways and conduits of communication.” Those pathways, moreover, rely on “specific residues in the structure” that contribute to the communication. The authors are clearly in awe of what they are seeing. What does the talk about “co-evolution” contribute, other than fanciful storytelling? Let the “logic of interaction and assembly” speak for itself.
Make Like Dynein: More Remote Control
In a recent article at Evolution News, remote control via water “tsunamis” was found in dynein, a molecular machine that walks on microtubules carrying cargo. A team at Rockefeller University found allosteric remote control in a “dynein-like” AAA protein named Mdn1, where AAA is short for “ATPase associated with diverse cellular activities,” and “ATPase” is any molecular machine using ATP for energy. There are about 100 AAA proteins in humans; many AAA proteins have a ring of six identical units at one end (the hexamer) where ATP is spent. In their paper in PNAS2, Mickolajczyk et al.describe Mdn1 as “an essential mechanoenzyme that uses the energy from ATP hydrolysis to physically reshape and remodel, and thus mature, the 60S subunit of the ribosome.” So here is another machine involved with the large (60S) part of the ribosome that has remote control to learn about.
Mdn1 is an unusually large enzyme, weighing in at over 500 kilodaltons, where a dalton is one atomic mass unit. This is a big machine, therefore, on the order of 500,000 hydrogen atoms in mass, with well over 5,000 amino acid residues in humans. A mechanoenzyme converts chemical energy into motion. The L-shaped enzyme looks something like a ring buoy (the AAA hexamer) with a back rest (the linker). Somehow this big device performs the job of removing assembly factor proteins from precursors for the 60S ribosomal subunit (for information on assembly factors, see this paper from the journal RNA). Surprisingly, though, about 1/10th of Mdn1’s amino acid residues form an “intrinsically disordered” domain — i.e., they do not fold into a permanent structure. Sounds interesting.
“This study provides insights into the regulation of Mdn1 function and into the design principles of large, multidomain mechanoenzymes,” the authors begin. They don’t know “why Mdn1 has remained a single polypeptide, and one of the largest enzymes in the genome, throughout eukaryotic evolution,” but spend no more time on that question. What they do look for is evidence of allosteric interactions — remote control — in the enzyme. Figures in the paper show Mdn1’s disordered region looks like a tether. It attaches to the top of the linker at one end and connects to its “MIDAS” domain (“metal ion-dependent adhesion site”) on the other end. The MIDAS domain docks to the ring buoy, then attaches to an assembly factor on the “pre-ribosome” (a large surface) and pulls it off, using the tether. It sounds like a relatively simple operation. Why such a big machine?
The authors do not come to firm conclusions about this essential machine’s mode of action but suggest that the enzyme is sharing information between its parts.
Mdn1 may not follow the canonical AAA mechanism of threading substrates through the central pore of its hexameric AAA ring. Here, we extend existing models to suggest that Mdn1 instead uses its MIDAS domain to (i) simultaneously bind to both an assembly factor and its own AAA ring, (ii) transmit information about substrate binding (mimicked by Rbin-1) to the AAA ring (Figs. 4 and 5), and (iii) transmit force produced in the AAA ring to the assembly factor. This MIDAS-mediated mechanism may be a more general alternative strategy utilized by a subclass of AAA proteins.
It sounds like there is a lot more to learn about Mdn1. Suffice it to say that the machine does more than attach and pull on things; it transmits information and force through remote controls. To see why such a large machine is needed to remove assembly factors for the ribosome, consider this paragraph from that paper in RNA cited earlier3:
Assembly of ribosome, the molecular machine for protein synthesis, is a fundamental and highly complex processthat involves transcription, modification, and processing of ribosomal RNAs (rRNAs) and assembly of ribosomal proteins. More than 200 assembly factors (AFs) and many snoRNAs participate in ribosome assembly (Henras et al. 2008; Kressler et al. 2010; Woolford and Baserga 2013). A number of preribosomal particles are formed as the small 40S and large 60S subunit mature in the nucleolus, the nucleoplasm, and the cytoplasm.
All these are necessary for a machine that translates proteins. This sounds like a huge chicken-and-egg problem; the ribosome needs all these assembly factors and subunits, but they need the ribosome to exist.
Allostery in the Nucleosome
Here is one more example of allostery to briefly consider. Before cell division, the genome needs to be compacted into dense supercoils to form the familiar chromosomes. Coiling occurs around nucleosomes, which consist of histone proteins, which act like spools for DNA to wrap around. In another PNAS paper4, Tan and Takada found a “long-distance” allosteric effect between the nucleosome and two transcription factors. They see Boolean logic in allostery:
Upon binding of effectors, allosteric molecules change their structures and responses to the downstream molecules, which can be viewed as the molecular if−then device. Simulating binding of two pioneer transcription factors (TFs), Sox2 and Oct4, to a nucleosome, which is the fundamental unit of genome folding, we found that a nucleosome acts as a new type of allosteric molecule. Free nucleosomes exhibited rotation-coupled sliding of their DNA among metastable positions. The Sox2 binding on them selected a specific rotational phase of its motif, inducing global sliding of nucleosomal DNA. Consequently, the repositioned DNA affected the accessibility of another TF, Oct4, or the second molecule of Sox2 at a distant region within the nucleosome, which thus is a long-distance allosteric effect.
No Hesitation
If anyone found a machine that operated another machine by remote control, they would not hesitate to conclude design. This happens in cells at the molecular scale but is the same principle. Molecular machines are already dazzling examples of intelligent design by reason of their precise functions. To find that many of them also act as remote control devices that transmit information and force over long distances should amplify the design inference beyond reasonable doubt.
Notes
- Walker et al., “RNA sectors and allosteric function within the ribosome.” PNAS, August 3, 2020. DOI: 10.1073/pnas.1909634117.
- Mickolajczyk et al., “Long-range intramolecular allostery and regulation in the dynein-like AAA protein Mdn1.” PNAS, August 4, 2020; 117 (31) 18459-18469. DOI: 10.1073/pnas.2002792117.
- Hu, Zhu and Ye, “Structure and RNA recognition of ribosome assembly factor Utp30.” RNA, 2017 Dec; 23(12): 1936–1945. DOI: 10.1261/rna.062695.117.
- Cheng Tan and Shoji Takada, “Nucleosome allostery in pioneer transcription factor binding.” PNAS, August 10, 2020. DOI: 10.1073/pnas.2005500117.