Evolution
Intelligent Design
Muller Two-Step Model: A Refutation of Behe on Irreducible Complexity?
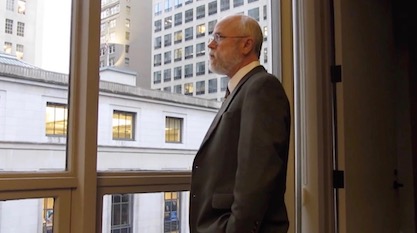
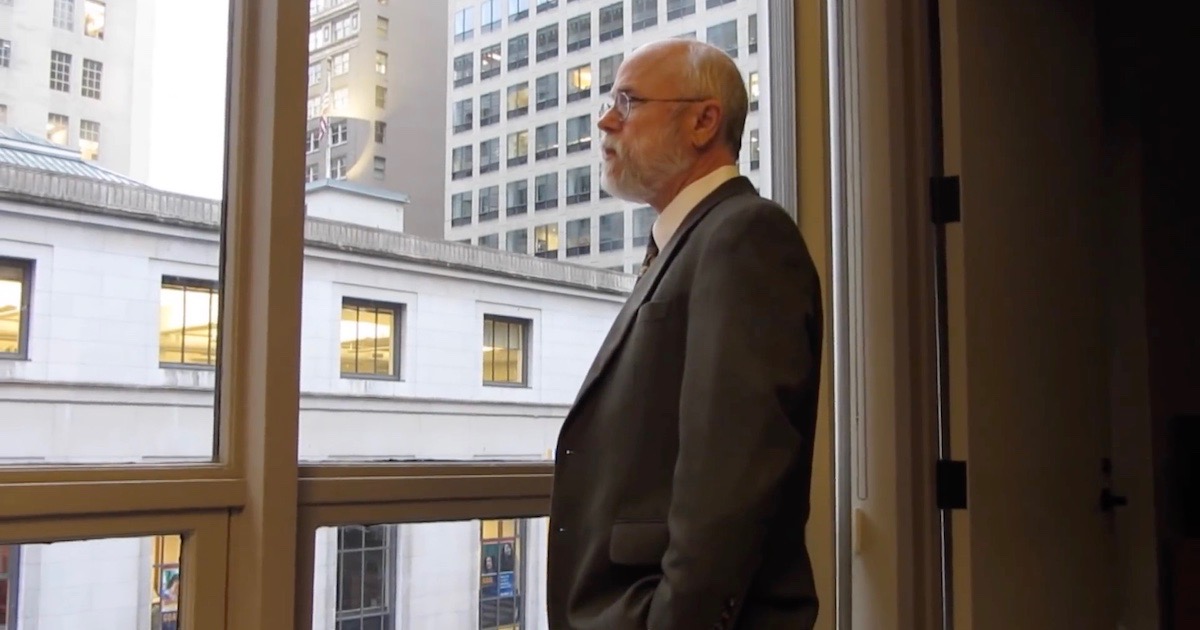
In his article in Skeptic Magazine reviewing Michael Behe’s new book, Darwin Devolves, biologist Nathan Lents raises a number of objections to Behe’s arguments. Many of those have already been addressed here and here. One of his objections was also brought up by Joshua Swamidass in a recent online discussion. This is the argument concerning the Muller two-step model, named after geneticist Hermann Joseph Muller, for explaining irreducibly complex features (Muller, 1918; Muller, 1939). This argument has been advanced several times in the past, including by Douglas Theobald and Alan Orr.
As Lents writes: “In 1918, H. J. Muller first proposed his two-step model of evolutionary innovation: ‘Add a part; make it necessary,’ which pre-empted Behe’s theory of irreducible complexity by 80 years and remains unchallenged.” Some ID critics (and perhaps even some ID proponents) who are relatively new to this debate may think that this is a promising objection to ID. However, it has actually been answered by ID proponents numerous times, in numerous different ways. For starters, we’ve addressed the Muller two-step model multiple times at Evolution News, including here, here, and here.
Muller’s Basic Concept
The basic concept is that an irreducibly complex system can evolve gradually by successive adding of parts that are, to begin with, only helpful but non-essential. Subsequently they are rendered essential by modification or loss of other components. As far back as 1997, Alan Orr summarized:
Molecular evolutionists have shown that some genes are duplications of others. In other words, at some point in time an extra copy of a gene got made. The copy wasn’t essential — the organism obviously got along fine without it. But through time this copy changed, picking up a new, and often related, function. After further evolution, this duplicate gene will have become essential. (We’re loaded with duplicate genes that are required: myoglobin, for instance, which carries oxygen in muscles, is related to hemoglobin, which carries oxygen in blood. Both are now necessary.) The story of gene duplication — which can be found in every evolution text — is just a special case of Muller’s theory. But it’s an immensely important case: it explains how new genes arise and, thus, ultimately, how biochemical pathways get built.
Two years later, in 1999, the National Academy of Sciences published one of its first official condemnations of ID and irreducible complexity. As they said, “The evolution of complex molecular systems can occur in several ways. Natural selection can bring together parts of a system for one function at one time and then, at a later time, recombine those parts with other systems of components to produce a system that has a different function. Genes can be duplicated, altered, and then amplified through natural selection.” An article by Theobald at the TalkOrigins website describes the Muller two-step model as follows:
- Add a part.
- Make it necessary.
The article promises that this explanation shows why “‘irreducible complexity’ is silly.” But its frank and candid description of the “Muller two step” shows why this model is unable to build new complex biological features. Indeed, ID proponents have shown for years why it does not work and why it hardly solves the challenge posed by irreducible complexity. To find out why, read on.
Defining Irreducible Complexity

Irreducible complexity is properly understood as a property of a system that is contributed to by multiple subfunctions, the removal of one of which causes the overall system to effectively cease performing its job. Note that each individual subfunction could, in principle, be performed by multiple protein components. Likewise, a single protein component could perform more than one of those subfunctions. Furthermore, the identity of the specific components performing each respective subfunction could differ from one organism to the next.
To illustrate, consider an analogy. In Darwin’s Black Box, Behe put forward the mousetrap as an everyday example of an irreducibly complex system (Behe, 1996). The mousetrap is composed of at least five component parts: the platform, the spring, the hammer, the hold-down bar, and the catch. One may add to this list the staples that are required to hold the trap together. If any one of these parts is removed, the mousetrap will no longer perform its function of catching mice. But to extend the analogy a bit further, imagine that the spring became duplicated such that the mousetrap came to possess an extra spring. This spring is not essential. Nonetheless a second spring does make the trap suitable for catching rats, which are bigger than mice. It therefore does provide an advantage. Now imagine that the original spring became modified or even lost in some way and that this rendered the second spring (which was originally only helpful) essential. Thus, we have changed the identity of the component performing the job of the spring. Still, the presence of a component (or components) performing this job is essential to the overall function of the trap.
Once one appreciates irreducible complexity, one immediately understands why it won’t work to “add a part” (or one could say “add a function”) and then “make it necessary.” Irreducibly complex features are made of multiple interacting parts (or functions). Simply adding a part (or function) to a complex system gives you no guarantee that it will interact with that system, much less interact in a way that will improve the function of the system rather than harming it. Similarly, throwing a monkey wrench into a machine will certainly add a part, but not in a way that makes it useful or necessary. As Michael Behe points out in Darwin Devolves, the vast majority of possible functional changes to a system tend to screw it up. Simply throwing parts at biological systems in no way makes it likely that those parts will be useful or beneficial. Much more likely the part will be useless, in which case they it will likely be selected out as a waste of resources, or harmful, in which case it will be quickly deleted by purifying selection.
The Case of Bacterial Cell Division
To illustrate these points further, let us take a biological example. Consider the case of bacterial cell division. The FtsZ ring, which assembles at the division septum, has to be tethered to the inner membrane. In the gamma-proteobacteria (e.g., Escherichia coli and Salmonella enterica), this is accomplished by the proteins ZipA, FtsA and ZapA (Pichoff and Lutkenhaus, 2002; Hale et al., 2011; Huang et al., 2013). ZipA and FtsA are essential, whereas ZapA contributes to the efficiency of the process but is not essential (Hale et al., 2011). As it turns out, ZipA (despite being essential among the gamma-proteobacteria) is not found outside the gamma-proteobacteria, and it has been reported that “the requirement for ZipA can be bypassed completely by a single alteration in a conserved residue of FtsA” (Geissler et al., 2003). Evidence also indicates that increased concentrations of FtsA can compensate for a lack of ZipA. For instance, FtsA is present in significantly higher concentrations in the Firmicute bacterium Bacillus subtilis than in Escherichia coli (Feucht et al., 2001).
This example serves as a good case study for the sort of evolutionary scenario envisioned by Lents, Orr, Theobald, and others. Presumably, ZipA was at first useful to the Gamma-proteobacteria, but subsequently came to be made essential by a modification or decrease in concentration of FtsA.
However, while any given anchor protein, such as ZipA, is not essential for successful cell division, at least some protein fulfilling this job is required. Nonetheless, a strong case may be made that this system is irreducibly complex. For, unless FtsZ and a tether are present together, the system is useless. What sense is there in having the tether without FtsZ for it to anchor to the inner membrane? And what sense is there in having FtsZ without having a protein to anchor it to the inner membrane? It seems difficult to envision a plausible evolutionary route for the origins of such a system.
Gene Duplication and the Evolution of the Globins
It would be interesting to see biological examples that do not fit this paradigm, of simply replacing proteins performing a given function with other proteins performing the same function. Readers will recall the example offered by Alan Orr above, namely, that of the relationship of haemoglobin to myoglobin:
We’re loaded with duplicate genes that are required: myoglobin, for instance, which carries oxygen in muscles, is related to hemoglobin, which carries oxygen in blood. Both are now necessary.
This argument too is echoed by the National Academy of Sciences’ 1999 response to ID, where (perhaps borrowing arguments from Orr) it noted sequence similarities between myoglobin and haemoglobin and argued: “It was immediately obvious in 1959 that the two molecules are very closely related.”
There are quite a few problems with this argument. One is that Orr and the NAS have merely asserted, not demonstrated, that the similarities between myoglobin and haemoglobin are the result of blind and mindless mechanisms. Like many ID critics today, Orr and the NAS provide potential evidence of common ancestry but they do not provide evidence of natural selection. And as Michael Behe reminded us back in 1997 in his response to Orr, mere sequence similarity may provide evidence of a common origin, but evidence of common ancestry is not a demonstration of a Darwinian evolutionary pathway:
Orr turns to the topic of gene duplication: “So how does Behe explain duplicate genes? He doesn’t.” But I do. I discuss them on pages 89-90 of my book, concluding “The sequence similarities are there for all to see…. By itself, however, the hypothesis of gene duplication…says nothing about how any particular protein or protein system was first produced.” For example, the DNA in each of the antibody-producing cells of your body is very similar to that of the others, but not identical. The similarities are due to common descent; that is, all the cells in your body descended from one fertilized egg cell. The differences, however, are not due to Darwinian natural selection. Rather, there is a very clever, built-in program to rearrange antibody genes. Billions of different kinds of antibody genes are “intentionally” produced by your body from a pre-existing stock of just a few hundred gene pieces. Perhaps because of his unfamiliarity with molecular systems, Orr has trouble seeing that similarity in gene sequences may indicate common ancestry, but is not itself evidence that a system was constructed by natural selection.
To test natural selection requires much more evidence than mere sequence similarity: it requires experimentation. In all of the scientific literature, however, no experimental evidence can be found that natural selection can produce irreducibly complex biochemical systems. To rebut my arguments Orr could simply have cited papers in the science literature where the systems I discuss have been explained. He didn’t do that because explanations are nowhere to be found.
Stepwise Explanations Don’t Work
There are good reasons why there aren’t published evolutionary accounts of the Darwinian origin of such complex molecular features: in these types of cases stepwise evolutionary explanations simply don’t work. According to a published bacterial population model, the model of gene duplication followed by recruitment only works if few modifications are required in order to acquire a new function (Axe, 2010). According to this model, if a duplicated gene is neutral (incurring no fitness cost), then the number of mutations necessary to acquire the new function (exclusive of the duplication event itself) cannot exceed six. If (more realistically) the duplicated gene incurs a slight fitness cost, then the maximum number of mutations cannot exceed two. Bacterial populations are far more massive than animal population sizes, and their generation turnover times are far shorter. Therefore, the process is going to be even more difficult in animal populations.
A third problem with employing blind mechanisms to make new parts useful in a system is that the change in primary sequence (to yield a new function for the protein) must be accompanied by alterations to the regulatory sequences controlling the expression of the gene. Let’s envision that the gene coding for haemoglobin became duplicated and that one of the duplicate copies retained its original function of carrying oxygen in the blood, while the other evolved into myoglobin. Myoglobin has a stronger binding affinity for oxygen than haemoglobin, and it helps extract oxygen from the blood for use by the muscle. It is not enough, however, to simply modify the amino acid sequence of haemoglobin in order to convert it to myoglobin. There would also have to be accompanying modifications to the gene’s regulatory sequences such that the protein was expressed in the muscle rather than in bone marrow where red blood cells are formed. No selective advantage would be served by having red blood cells containing myoglobin. In fact, it would be deleterious to the organism’s fitness since the oxygen would be bound too tightly and not released to the tissues. Thus, the difficulty of changing one protein into another is often compounded by the requirement for accompanying modifications to the regulatory sequences that control gene expression.
Conclusion
While the Muller two-step scenario is a clever idea, for the reasons given above it is highly unlikely that it can account for systems that require the performance of multiple subfunctions in order to work. Our responses to the Muller two-step have been around for a long time; it would be nice if ID critics would recognize them and perhaps even answer them rather than inaccurately proclaiming that their arguments go “unchallenged.”
Literature Cited
Axe, D. (2010) The Limits of Complex Adaptation: An Analysis Based on a Simple Model of Structured Bacterial Populations. Bio-complexity 2010.
Behe, M.J. (1996) Darwin’s Black Box. Free Press.
Feucht, A., Lucet, I., Yudkin, M.D., Errington, J. (2001) Cytological and biochemical characterization of the FtsA cell division protein of Bacillus subtilis. Molecular Microbiology 40(1):115-125.
Geissler, B., Elraheb, D. and Margolin, W. (2003) A gain-of-function mutation in ftsA bypasses the requirement for the essential cell division gene ZipA in Escherichia coli. Proceedings of the National Academy of Sciences USA 100(7):4197-4202.
Hale, C.A., Shiomi, D., Liu, B., Bernhardt, T.G., Margolin, W., Niki, H. and de Boer, P.A.J. (2011) Identification of Escherichia coli ZapC (YcbW) as a Component of the Division Apparatus That Binds and Bundles FtsZ Polymers. Journal of Bacteriology 193(6):1393-1404.
Huang, K.H., Durand-Heredia, J. and Janakiraman, A. (2013) FtsZ Ring Stability: of Bundles, Tubules, Crosslinks, and Curves. Journal of Bacteriology 195(9):1859-1868.
Muller, H. J. (1918) “Genetic variability, twin hybrids and constant hybrids, in a case of balanced lethal factors.” Genetics 3:422-499.
Muller, H. J. (1939) “Reversibility in evolution considered from the standpoint of genetics.” Biological Reviews of the Cambridge Philosophical Society 14:261-280.
Pichoff, S. and Lutkenhaus, J. (2002) Unique and overlapping roles for ZipA and FtsA in septal ring assembly in Escherichia coli. The EMBO Journal 21(4):685-693.
Photo: Michael Behe, a scene from Revolutionary: Michael Behe and the Mystery of Molecular Machines.